The road to Bose–Einstein condensates and degenerate Fermi gases was paved with ideas that shouldn’t have worked, but did, as Chad Orzel explains in the final segment of his three-part history of laser cooling. Read part one and part two first
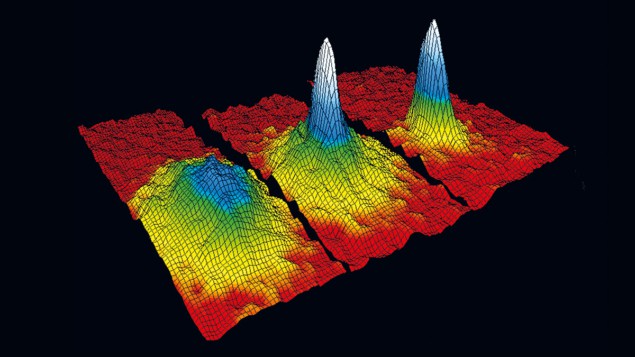
During the last two decades of the 20th century, atomic physicists repeatedly broke the record for the coldest temperature in the universe. These achievements rested on a handful of advances, including laser cooling (as described in part 1 of this history), the magneto-optical trap and techniques such as Sisyphus cooling that worked better than expected (as described in part 2). By 1990, physicists were routinely cooling tens of millions of atoms to temperatures a few tens of microkelvin above absolute zero – a thousand times colder than conventional cryogenics and a fraction of the “Doppler cooling limit” predicted for laser-cooling simple atoms.
As dramatic as this plunge was, though, an even more challenging drop in temperature beckoned: a further factor of 1000, from microkelvin to nanokelvin. This additional drop would introduce a new realm of physics known as quantum degeneracy. Here, low temperatures and high densities force atoms into one of two exotic states of matter: either a Bose–Einstein condensate (BEC), in which all of the atoms in a gas coalesce into the same quantum state, or a degenerate Fermi gas (DFG), in which the total energy of the gas stops decreasing because all the available energy states are full (figure 1).
BECs and DFGs are purely quantum phenomena, and an atom’s total spin dictates which of them will form. If the atom has an even number of electrons, protons and neutrons, it’s a boson and can undergo BEC. If the total is odd, it’s a fermion and can make a DFG. Different isotopes of the same element sometimes behave in opposite ways – physicists have made BECs of lithium-7 and DFGs with lithium-6 – and this difference in low-temperature behaviour is one of the most dramatic demonstrations of the fundamental division between quantum particles.
1 Quantum statistics in action

At high temperatures, both bosons (blue dots) and fermions (green dots) are distributed across a wide range of the available energy states. When released from a trap, they expand outward to form a spherical cloud with a width that reflects their temperature. As the atoms are cooled, they shift to lower energy states and the size of the cloud decreases. However, whereas bosons can have multiple atoms in the same state, fermions can have only a single atom in each state. Below some critical temperature, this fact leads nearly all the bosons to collect in a single energy state, forming a Bose–Einstein condensate, which shows up as a small and very dense clump in the centre of the cloud. In a degenerate Fermi gas, on the other hand, all the low-energy states are filled, so the cloud cannot shrink further. The experimental images in the centre of this diagram show clouds of bosonic (left) and fermionic (right) lithium atoms behaving differently as they are cooled. Here, TF is the Fermi temperature, which marks the onset of quantum degeneracy in fermions.
As with previous breakthroughs described in this series, the dive to quantum degeneracy came about thanks to new technologies introduced in research labs scattered around the world. And – again as with the earlier advances – one of these technologies arrived entirely by chance.
Laser cooling on the cheap
In the mid-1980s, Carl Wieman was studying parity violation in caesium atoms at the University of Colorado, Boulder, in the US. These studies require time-consuming and exacting spectroscopy measurements, and Wieman’s PhD student Rich Watts developed a way to do them using diode lasers like the ones being manufactured by the million for CD players.
After spending years figuring out how to stabilize and control these cheap, solid-state devices, Watts (quite reasonably) wanted to finish his PhD, so he and Wieman looked around for a shorter-term experiment to test them. The answer they hit upon was laser cooling. “It was this fun little side thing to finish off this student’s thesis,” recalls Wieman, “and that’s completely how I got into [laser cooling].”
In 1986 Watts and Wieman became the first to laser cool a beam of caesium atoms. Watts was also the first to laser cool rubidium, as a postdoc with Hal Metcalf at Stony Brook University in New York, and he participated in the seminal experiments that revealed sub-Doppler cooling in Bill Phillips’ lab at the US National Institute of Standards and Technology (NIST) in Gaithersburg, Maryland. However, like another key player we will meet in this history, Watts left the stage too soon, dying aged just 39 in 1996.
Wieman, meanwhile, needed a new scientific target, something that could only be done with cold atoms. He, along with new colleagues and competitors, found it in a very old idea with an impeccable scientific pedigree: Bose–Einstein condensation.
A race to the bottom
In 1924 Satyendra Nath Bose was a physicist at the University of Dhaka in what is now Bangladesh. While teaching the new and rapidly developing field of quantum physics, he realized that Max Planck’s formula for the spectrum of light from a hot object could be derived from the statistical rules governing the behaviour of photons, which are far more likely than classical particles to be found in the same states.

Bose had trouble getting his work published, so he sent a copy to Albert Einstein, who loved it so much he arranged for it to be published in Zeitschrift für Physik alongside a paper of his own. Einstein’s contributions included extending photon statistics to other types of particles (including atoms) and pointing out an interesting consequence: at very low temperatures, the most likely state of the system is for all the particles to occupy the same energy state.
This collective state is now called a BEC and is closely related to superfluidity and superconductivity, which are observed in liquids and solids (respectively) at temperatures near absolute zero. The BEC transition itself, though, could in principle occur in a dilute gas of atoms – just like the ones atomic physicists began creating in the 1970s.
There were a few barriers, though. One is that the critical temperature at which a BEC forms is determined by density: the lower the density, the lower the critical temperature. Although Sisyphus cooling made microkelvin temperatures possible, laser-cooled atomic vapours are so diffuse that their transition temperature is even lower, in the nanokelvin range. It is also lower than the “recoil temperature” associated with atoms absorbing or emitting a single photon. Cooling below this limit must therefore be done without lasers.
One evaporation at a time
The general solution to these problems came from Daniel Kleppner and colleagues at the Massachusetts Institute of Technology (MIT). It is similar to the mechanism that cools a cup of tea. The water molecules in the tea move at different speeds, and the fastest have enough energy to break free and float away as water vapour. Because these “escapees” carry a greater-than-average amount of energy, the remaining molecules end up colder. Once the energy in their motion is redistributed through collisions between molecules, the system reaches a new equilibrium at a lower temperature (figure 2).
Kleppner’s method is known as evaporative cooling, and it requires two elements: a means of selectively removing the hottest atoms from the trap, and a rate of collisions between atoms that is high enough for the sample to re-equilibrate afterwards. The first element came hand-in-hand with the solution to the photon recoil problem: atoms can be kept “in the dark” by transferring them from a magneto-optical trap (MOT) to a purely magnetic trap like the one Phillips first made in 1983. The higher energy of the “hot” atoms requires a larger magnetic field to confine them, and this large magnetic field produces a Zeeman shift in the atoms’ energy levels. A properly tuned radio-frequency signal can thus flip the “hot” atoms at this high field into an un-trapped state without disturbing the colder ones. The colder atoms left behind are also restricted to a smaller volume, so as the temperature decreases the density increases, bringing the system closer to BEC in two ways.
2 How low can you go

Evaporative cooling works by removing the highest energy atoms (red) from a trapped vapour containing a large number of atoms distributed across the available energy states in the trap. The atoms left behind will undergo collisions that redistribute the total energy among the atoms. Although some of them will gain energy (orange), the average energy (and thus the temperature) will be lower, as indicated by the dashed lines. This process of removing hot atoms and redistributing energy is then repeated, lowering the temperature further.
The collision issue, however, is out of experimentalists’ hands. The relevant rate is described by a single parameter: the so-called scattering length for a pair of colliding atoms in particular states. If this scattering length is moderately large and positive, the evaporation will proceed rapidly and the resulting condensate will be stable. If the scattering length is too small, the evaporation will be very slow. If it is negative, the condensate will be unstable.
The obvious solution is to pick an atom with the right scattering length, but this parameter turns out to be exceedingly difficult to calculate from first principles. It needs to be determined empirically, and in the early 1990s no-one had done the necessary experiments. Consequently, the groups that began pursuing BEC chose different elements from the periodic table, each hoping that “theirs” might turn out to be “right”. Wieman and his new colleague Eric Cornell even switched from caesium to rubidium because rubidium’s two stable isotopes doubled their chances.
“That’ll never work”
Because a MOT can be turned into a purely magnetic trap simply by switching off the lasers and running more current through the magnet coils, the first steps toward BEC were a straightforward extension of laser-cooling experiments. The resulting “quadrupole trap” configuration has just one major problem: the field in the centre of the trap is zero, and at zero field, atoms can change their internal states to one that is not trapped any more. Plugging this “leak” of atoms from the trap centre requires finding a way to keep the trapped atoms from changing states.
For several years, this was a major area of laser-cooling research. In addition to Cornell and Wieman, one of the main contenders in the intensifying BEC race was Wolfgang Ketterle of MIT. His group developed a way of pushing atoms away from the zero-field region using a blue-detuned laser focused on the centre of the trap as a “plug”. Cornell and Wieman, for their part, used an all-magnetic technique they called a time orbiting potential (TOP) trap.
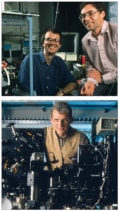
Cornell developed the TOP on a flight back from a conference in early 1994, motivated in part by the need to limit disruption to their apparatus. Though he and Wieman didn’t have room for another laser beam, they could add a small extra coil around an axis perpendicular to the quadrupole coils, and that would shift the zero-field position. Atoms in the trap would move toward the new zero, of course, but not quickly. If they used two small coils on different axes driven by oscillating currents to move the zero in a circle a few hundred times per second, that might be enough to keep it, in Cornell’s words, “everywhere where the atoms aren’t”.
They tested the idea that summer, using a small coil driven by a cheap audio amplifier. At first, the added field made the coils wound around their glass vapour cell rattle alarmingly, and the driven coils made a piercing, high-pitched whine, but the principle was sound, so they built a sturdier version. A few months later, in early 1995, Cornell discussed trap schemes with Ketterle, and came away thinking that the MIT team’s optical plug was “never going to work. It’s basically gonna be a big old swizzle stick pointing in there.” However, he acknowledges that Ketterle may have felt the same about the TOP: “He’s probably thinking ‘That’s the stupidest idea I ever heard in my whole life.’ So we both went away very satisfied from that conversation.”
As it happened, both techniques did, in fact, work. Cornell and Wieman were the first to demonstrate this, performing a series of experiments in which they shone a laser beam through their cold atom cloud. During these “snapshots”, atoms in the cloud would absorb photons from the laser, leaving a shadow in the beam. The depth of this shadow was a measure of the density of the cloud, while the size of the cloud indicated the temperature of the atoms. As the evaporation progressed, the snapshots showed a spherically symmetric cloud of atoms slowly shrinking and cooling as hot atoms were progressively removed.
Then, in June 1995, at a temperature of around 170 nanokelvin, something dramatic happened: a small dark spot appeared at the centre of their images, representing atoms at a drastically lower temperature and higher density. Cornell says it didn’t take long to work out what was going on: “The central density just shoots up. What’s happening there if not Bose–Einstein condensation?”
To confirm their suspicions, he and Wieman converted some of their shadow images into the now-iconic three-dimensional plots (see “The coolest result” image) showing the thermal atoms as a broad pedestal and the BEC as a “spike” emerging in the centre. The shape of the spike – wider in one direction than the other – encoded a clue. Because their TOP trap was stronger in the vertical direction than the horizontal, the condensate was squeezed more tightly in that direction, meaning it expanded more rapidly in that direction after release. While they had not predicted this shape change, they were quickly able to explain it, adding to their confidence that they had reached the “holy grail” of BEC.
Cornell and Wieman announced their results (unusually, for those days) in a press conference in early June 1995. Their paper was published in Science the following month. In September, Ketterle and colleagues produced their own set of 3D plots showing a similar “spike” emerging as their cloud of sodium atoms reached the transition temperature. Cornell, Wieman and Ketterle went on to share the 2001 Nobel Prize for Physics for the achievement of BEC in dilute atomic vapours.
Fermions get their champion
In the early months of 1995, Cornell recruited a new postdoc, Deborah “Debbie” Jin. Her husband John Bohn, a physicist at NIST in Boulder, recalls Cornell saying, “A lot of people will tell you that BEC is still years off, but I really think we’re going to do it.” He was right: the first BEC happened between the time Jin agreed to take the job and when she started work.
Jin came from a different research community – her thesis was on exotic superconductors – but she quickly learned about lasers and optics, and played a key role in early experiments probing the properties of BEC. As a rising star, she had numerous offers of a permanent position, but she elected to stay at JILA, a hybrid institution that combines expertise from the University of Colorado and NIST. There, to distinguish her work from that of Cornell and Wieman, she decided to pursue the other class of ultra-low-temperature behaviour: degenerate Fermi gases.
Where bosons are governed by statistical rules that make it more likely for two of them to be found in the same energy state, fermions are absolutely forbidden from sharing states. Applied to electrons, this is the Pauli exclusion principle that explains much of chemistry: electrons in an atom “fill up” the available energy states, and the exact state of the last electrons determines the chemical properties of a given element. Fermionic atoms in a magnetic trap obey a similar rule: as the gas is cooled, the lowest states fill up. At some point, though, all of the low-energy states are full, and the cloud can’t shrink any further. As with BEC, this is a purely quantum phenomenon, having nothing to do with interactions between the particles, so it should be observable in a gas of ultracold atoms.

Jin started at JILA in 1997 with a single graduate student, Brian DeMarco, who had been hired by Cornell but switched to work with Jin on Cornell’s recommendation. As DeMarco recalls, Cornell told him, “If you and Debbie can be the first people to make a DFG, it’ll be a big deal, and there’s a good shot of doing it.”
The pair began with an empty lab, lacking even furniture. Bohn recalls them sitting on the floor in the office he shared with Jin, assembling electronics for their future lasers. Within a year, though, they had a working apparatus for magnetic trapping and evaporatively cooling fermionic potassium atoms.
The quest for a DFG poses two challenges beyond those faced in the BEC race. The first of these is that at ultra-low temperatures, the collisions needed for the re-equilibration step of evaporative cooling stop happening because the prohibition on two fermions being in the same state keeps them from colliding. To solve this, Jin and DeMarco placed half their atoms in a different internal state, providing enough cross-state collisions to enable evaporation. At the end of the process, they could remove one of the two states and image the rest.
The second issue is that while the experimental signature of BEC is a giant density spike in the middle of the atomic cloud, Fermi degeneracy is more subtle. The key phenomenon of atoms refusing to clump together manifests itself undramatically in the form of the cloud ceasing to shrink further once the transition temperature is reached. Working out how to distinguish the degenerate gas from the thermal cloud took careful modelling and an imaging system that could reliably measure tiny changes in the shape of the distribution.
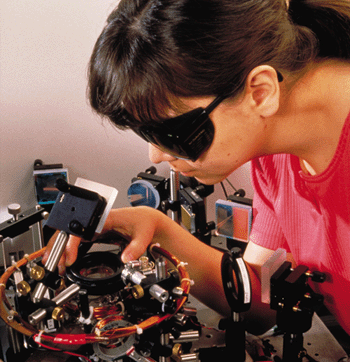
A Fermi gas of atoms
Despite these challenges, a mere 18 months after starting with an empty room, Jin and DeMarco published the first observation of a degenerate Fermi gas. A few years later, teams led by Ketterle, Randy Hulet at Rice University, Christophe Salomon at ENS in Paris, and John Thomas at Duke University, followed.
Jin, meanwhile, went on to use lasers and magnetic fields to convert degenerate atoms into molecules, opening new frontiers in ultracold chemistry. This work attracted numerous accolades, including a MacArthur Foundation “genius grant”, the I I Rabi Prize from the American Physical Society (APS) and the Isaac Newton Medal of the Institute of Physics. Jin would’ve been a shoo-in for yet another Nobel prize in ultracold-atom physics, too, but alas, she died of cancer in 2016, and the prize is not awarded posthumously.
Beyond prizes, though, Jin’s legacy is substantial. The sub-field she started has grown into one of the most important areas of atomic physics, and her former students and colleagues continue to lead the study of ultracold fermions. In recognition of her commitment to mentoring, the APS created an annual Deborah Jin Award for Outstanding Doctoral Thesis Research in Atomic, Molecular or Optical Physics.
A history of ongoing discovery
This series covers a little more than half a century. During that time, the idea of using lasers to manipulate atoms went from an idle curiosity in the mind of a single Bell Labs physicist to a foundational technique for a vast swathe of cutting-edge physics. Laser-cooled ions are now one of the most important platforms for the development of quantum information science. Laser-cooled neutral atoms provide the basis for the world’s best atomic clocks. And the quantum degenerate systems first observed by Cornell, Wieman, Ketterle and Jin spawned a huge sub-field that connects atomic physics to condensed-matter physics and chemistry. Laser-cooled atoms continue to be vital for physics research, and new history is being written daily in labs around the world.
- SEO Powered Content & PR Distribution. Get Amplified Today.
- PlatoData.Network Vertical Generative Ai. Empower Yourself. Access Here.
- PlatoAiStream. Web3 Intelligence. Knowledge Amplified. Access Here.
- PlatoESG. Carbon, CleanTech, Energy, Environment, Solar, Waste Management. Access Here.
- PlatoHealth. Biotech and Clinical Trials Intelligence. Access Here.
- Source: https://physicsworld.com/a/coldest-how-a-letter-to-einstein-and-advances-in-laser-cooling-technology-led-physicists-to-new-quantum-states-of-matter/
- :has
- :is
- :not
- :where
- $UP
- 1
- 1994
- 1995
- 1996
- 2001
- 20th
- 39
- 3d
- 87
- a
- Able
- About
- above
- Absolute
- absolutely
- AC
- achievement
- achievements
- across
- add
- added
- adding
- addition
- Additional
- advances
- After
- afterwards
- again
- aged
- agreed
- aip
- All
- along
- alongside
- also
- Although
- American
- among
- amount
- an
- and
- announced
- annual
- Another
- answer
- any
- appeared
- applied
- archives
- ARE
- AREA
- areas
- around
- arranged
- arrived
- AS
- asking
- associated
- At
- atom
- atomic
- attracted
- audio
- available
- average
- award
- awarded
- away
- AXES
- Axis
- back
- Bangladesh
- barriers
- Basically
- basis
- BE
- Beam
- BEC
- became
- because
- been
- began
- behaviour
- behind
- being
- Bell
- below
- BEST
- Better
- between
- Beyond
- Big
- Blue
- boson
- both
- Bottom
- Break
- breakthroughs
- Bringing
- broad
- Broke
- built
- but
- by
- calculate
- called
- came
- CAN
- Cancer
- cannot
- careful
- Carl
- carry
- CD
- cell
- central
- centre
- Century
- challenges
- challenging
- Chance
- chances
- change
- Changes
- changing
- cheap
- chemical
- chemistry
- chose
- Circle
- class
- click
- Clocks
- closely
- closer
- Cloud
- coalesce
- coil
- cold
- colleague
- colleagues
- collect
- Collective
- collision
- Colorado
- combines
- commitment
- community
- competitors
- completely
- Conference
- confidence
- Configuration
- Confirm
- connects
- consequence
- Consequently
- continue
- contributions
- control
- conventional
- Conversation
- convert
- converted
- Cool
- cornell
- could
- Course
- Covers
- created
- Creating
- critical
- Cup
- curiosity
- Current
- cutting-edge
- daily
- Dark
- Dave
- Days
- deal
- Debbie
- decades
- decided
- decreases
- demonstrate
- depicting
- depth
- Derived
- described
- determined
- determines
- developed
- developing
- Development
- Devices
- dictates
- DID
- difference
- different
- differently
- difficult
- direction
- directly
- discussed
- Disruption
- distinguish
- distributed
- distribution
- dive
- Division
- do
- doing
- done
- doubled
- dramatic
- drastically
- driven
- Drop
- Duke
- duke university
- during
- Dying
- each
- Earlier
- Early
- einstein
- either
- elected
- Electronics
- electrons
- element
- elements
- emerges
- emerging
- enable
- encoded
- end
- energy
- enough
- ENS
- entirely
- Equilibrium
- eric
- Even
- EVER
- exacting
- Exotic
- Expand
- expanded
- expected
- experiment
- experimental
- experiments
- expertise
- Explain
- Explains
- extending
- extension
- extra
- faced
- fact
- factor
- far
- fastest
- felt
- few
- field
- Fields
- Figure
- fill
- filled
- final
- finding
- finish
- First
- first steps
- flight
- Flip
- Float
- Floor
- focused
- followed
- following
- For
- Force
- form
- formation
- Former
- forms
- formula
- found
- Foundation
- foundational
- fraction
- Free
- from
- Frontiers
- full
- fun
- fundamental
- further
- future
- Gain
- GAS
- General
- get
- getting
- giant
- gif
- gift
- given
- glass
- going
- good
- got
- governed
- governing
- graduate
- Green
- Group
- Group’s
- grown
- had
- Half
- hand
- handful
- Hands
- happened
- Happening
- Have
- having
- he
- heard
- help
- her
- here
- High
- higher
- highest
- him
- his
- history
- Hit
- hoping
- Horizontal
- HOT
- hottest
- How
- How To
- However
- HTML
- HTTPS
- huge
- hundred
- Hybrid
- i
- idea
- ideas
- Idle
- if
- illinois
- image
- images
- Imaging
- immediately
- importance
- important
- in
- included
- Including
- Increases
- indicated
- information
- Institute
- Institution
- intensifying
- interactions
- interesting
- internal
- into
- introduce
- introduced
- isotopes
- issue
- IT
- itself
- Job
- John
- jpg
- june
- just
- just one
- Keep
- keeps
- kept
- Key
- known
- lab
- laboratory
- Labs
- lacking
- large
- larger
- laser
- lasers
- Last
- later
- Law
- lead
- Leads
- learned
- leaving
- Led
- left
- Legacy
- Length
- letter
- levels
- Library
- Life
- light
- like
- likely
- LIMIT
- lines
- little
- Long
- looked
- looking
- Lot
- loved
- Low
- lower
- Lowering
- lowest
- made
- Magnetic field
- Main
- major
- make
- manufactured
- many
- Maryland
- massachusetts
- Massachusetts Institute of technology
- Matter
- max
- max-width
- May..
- meaning
- means
- Meanwhile
- measure
- measurements
- mechanism
- Meet
- mentoring
- mere
- Metcalf
- method
- Middle
- might
- million
- millions
- mind
- MIT
- modelling
- moderately
- molecular
- Month
- months
- more
- most
- motion
- motivated
- move
- much
- multiple
- must
- my
- National
- Near
- nearly
- necessary
- Need
- needed
- needs
- negative
- Neutral
- neutrons
- never
- New
- New technologies
- New York
- Newton
- nist
- nobel prize
- nothing
- now
- number
- numerous
- object
- observation
- obvious
- of
- off
- Offers
- Office
- Old
- on
- once
- ONE
- ones
- ongoing
- only
- onset
- open
- opening
- opposite
- Optical physics
- optics
- or
- Orange
- orbiting
- Other
- out
- outstanding
- own
- pair
- Paper
- parameter
- paris
- parity
- part
- participated
- particular
- People
- per
- performing
- periodic
- permanent
- phd
- phenomenon
- photo
- Photons
- PHP
- physical
- Physics
- Physics World
- pick
- pioneered
- Platforms
- plato
- Plato Data Intelligence
- PlatoData
- played
- player
- players
- plug
- plunge
- Point
- poses
- position
- positive
- possible
- potential
- predicted
- press
- previous
- principle
- principles
- prize
- prizes
- probably
- Problem
- problems
- proceed
- process
- Produced
- produces
- progressed
- progressively
- Prohibition
- properly
- properties
- protons
- provide
- providing
- Publication
- published
- Publishing
- purely
- pursue
- Pushing
- Quantum
- quantum information
- quantum particles
- quantum physics
- quest
- quickly
- quite
- rabi
- Race
- range
- rapidly
- Rate
- reached
- Reaches
- Read
- realized
- really
- realm
- recognition
- Recoil
- Recommendation
- record
- Red
- reflects
- refusing
- region
- Rejected..
- related
- release
- released
- relevant
- remaining
- remove
- Removed
- removing
- repeated
- REPEATEDLY
- representing
- require
- requires
- research
- respectively
- REST
- restricted
- resulting
- Results
- Revealed
- Rice
- right
- rising
- road
- Role
- Room
- routinely
- Rule
- rules
- running
- s
- same
- satisfied
- saying
- says
- scattered
- schemes
- Science
- scientific
- Second
- see
- segment
- sent
- September
- Series
- set
- several
- Shadow
- Shape
- Share
- shared
- sharing
- she
- shift
- Shortly
- shot
- should
- show
- showed
- Shows
- side
- sign
- Signal
- signature
- similar
- Simple
- simply
- single
- Sitting
- Size
- slow
- Slowly
- small
- smaller
- So
- sodium
- solution
- SOLVE
- some
- something
- sometimes
- soon
- Sound
- Spectroscopy
- Spectrum
- speeds
- Spending
- spike
- Spin
- Spot
- stabilize
- stable
- Stage
- standards
- stanford
- Star
- started
- Starting
- State
- States
- statistical
- statistics
- stay
- Step
- Steps
- Still
- Stop
- Stops
- straightforward
- stronger
- Student
- Students
- studies
- Study
- Studying
- substantial
- such
- summer
- Superconductivity
- switched
- system
- Systems
- table
- Take
- taken
- Target
- Tea
- Teaching
- teams
- technique
- techniques
- Technologies
- Technology
- tell
- tens
- test
- tested
- than
- Thanks
- that
- The
- the world
- their
- Them
- then
- There.
- therefore
- thermal
- These
- thesis
- they
- thing
- think
- Thinking
- this
- those
- though?
- thousand
- three-dimensional
- Through
- thumbnail
- Thus
- tightly
- time
- time-consuming
- times
- to
- together
- told
- too
- took
- top
- Total
- toward
- Transferring
- transition
- trapped
- trapping
- trouble
- tuned
- TURN
- Turned
- turns
- two
- types
- undergo
- Universe
- university
- upon
- us
- use
- used
- using
- Vast
- version
- vertical
- very
- very satisfied
- VIOLATION
- visual
- vital
- volume
- wanted
- was
- Water
- Way..
- ways
- we
- went
- were
- What
- What is
- when
- whereas
- which
- while
- WHO
- whole
- wide
- Wide range
- wider
- width
- will
- with
- within
- without
- words
- Work
- work out
- worked
- working
- working out
- works
- world
- world’s
- would
- written
- year
- years
- yet
- york
- You
- zephyrnet
- zero