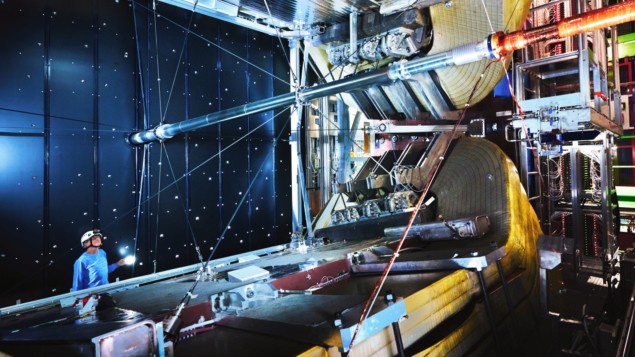
Physicists working on the LHCb experiment have seen evidence that “quark coalescence” plays a role in the evolution of quarks into hadrons following proton collisions at the Large Hadron Collider (LHC). This mechanism, which was originally proposed in the 1980s, has existing quarks with overlapping wavefunctions combining rather than creating new quarks. It is most pronounced at low transverse momenta, and gradually turns off as quarks escape rapidly from the collision point.
Quarks are the particles that make up the protons and neutrons inside atomic nuclei and numerous other hadrons (heavy particles) that feel the strong interaction. One of their strangest features is that they can never be observed in isolation. The main reason is that, unlike gravity, electromagnetism and the weak interaction, all of which drop in strength with distance, the effect of the strong interaction grows as bound quarks mover further apart. If quarks are sufficiently far apart, the gluon field that mediates the strong interaction contains enough energy to create particle-antiparticle pairs. These bind to the original quarks, creating new bound particles that can either be mesons (combinations of one quark and one antiquark) or baryons (comprising three quarks). This process is called fragmentation.
Experiments involving heavy ion collisions have suggested that this is not the whole story, however. Physicists believe that quarks can also combine in the dense quark–gluon plasma formed by smashing these large particles together in a process called coalescence.
“You have a collision, you make a bunch of quark–antiquark pairs that start moving away from each other, and because of wave-particle duality each particle has a wavelength that sort of tells you how big it is,” explains Matt Durham of Los Alamos National Laboratory in the US, who is a member of the LHCb collaboration.
Existing quarks combine
“If you have three quarks that overlap each other, you freeze them together into a baryon; if you have two quarks that overlap, you freeze them together into a meson; if you have a quark that doesn’t overlap with any other ones it has to fragment,” Durham explains. “So coalescence takes quarks that are produced in the collision and sticks them together; fragmentation requires you to make new quarks out of the vacuum.”
Coalescence in heavy ion collisions has been “generally accepted”, says Durham, because it is otherwise difficult to explain the ratios of protons to pions produced in experiments. Heavy ion collisions are messy, however, and theoretical predictions are inevitably imprecise. In the new research, the LHCb team studied the production of b quarks in proton-proton collisions. Sometimes called the bottom or beauty quark, the b quark is the second most massive quark in the Standard Model of particle physics.
The production of b quarks is almost certain to produce either a b-lambda baryon or a B0 meson, which both contain a b quark. The production ratio between these two has been extensively studied in experiments in which the b quark is produced by electron-positron collisions – a process that can lead only to fragmentation. “If you only have fragmentation, this ratio should be universal,” says Durham.
The LHCb team combed through several years’ data on proton–proton collisions and studied the decay products from collisions that had produced b quarks. For collisions with high transverse momenta relative to the colliding beams and few other outgoing particles detected at the same time, the baryon-to-meson ratio was approximately equal to the ratio in electron-positron experiments.
More baryons
However, as the transverse momenta dropped and as the number of other particles detected simultaneously grew, the proportion of baryons gradually increased relative to the proportion of mesons. This, the researchers concluded, was clear evidence that another process more likely to produce baryons was at work in these collisions. In this scenario the b quark is surrounded by other quarks – but became increasingly disfavoured as the produced quark was more separated from the other particles. “You really require coalescence to explain that,” says Durham, who adds, “I think we’ve shown it quite definitively here”.
Evidence grows for deconfined quark matter in neutron-star cores
“I definitely find the data convincing,” says theorist Ralf Rapp of Texas A&M University; “There used to be a disconnect between very small systems – the extreme being electron–positron, where you only have one quark–antiquark pair – and the heavy ion systems where you have thousands of quarks. The way they really make their point is to show systematically how the effect goes away and recovers the electron-positron limit as a function of how many hadrons are observed, which is an observable measuring how many quarks and antiquarks there are to coalesce with.”
Experimentalist Anselm Vossen of Duke University in North Carolina agrees that the work is “very nice”, but notes that the underlying assumptions used to calculate the fragmentation fractions involve the quarks being isolated, so it is perhaps unsurprising that they give incorrect results at low transverse momenta when this is not the case. “All these are models,” he says. “It’s very suggestive that if you use something in the coalescence model it works, but that doesn’t mean it’s ‘the truth’”
The research is described in Physical Review Letters.
- SEO Powered Content & PR Distribution. Get Amplified Today.
- PlatoData.Network Vertical Generative Ai. Empower Yourself. Access Here.
- PlatoAiStream. Web3 Intelligence. Knowledge Amplified. Access Here.
- PlatoESG. Carbon, CleanTech, Energy, Environment, Solar, Waste Management. Access Here.
- PlatoHealth. Biotech and Clinical Trials Intelligence. Access Here.
- Source: https://physicsworld.com/a/evidence-for-quark-coalescence-found-in-lhc-collisions/
- :has
- :is
- :not
- :where
- $UP
- 160
- a
- Adds
- ago
- agrees
- All
- almost
- also
- an
- and
- Another
- any
- apart
- approximately
- ARE
- AS
- assumptions
- At
- atomic
- away
- BE
- Beauty
- became
- because
- been
- being
- believe
- between
- Big
- bind
- both
- Bottom
- Bound
- Bunch
- but
- by
- calculate
- called
- CAN
- carolina
- case
- certain
- clear
- coalesce
- coalescence
- collaboration
- collision
- combinations
- combine
- combining
- comprising
- concluded
- contain
- contains
- Core
- create
- Creating
- data
- definitely
- described
- detected
- difficult
- distance
- Doesn’t
- Drop
- dropped
- Duke
- duke university
- durham
- each
- effect
- either
- energy
- enough
- equal
- escape
- evidence
- evolution
- existing
- experiment
- experiments
- Explain
- Explains
- extensively
- extreme
- far
- Features
- feel
- few
- field
- Find
- following
- For
- formed
- found
- fragmentation
- Freeze
- from
- function
- further
- Give
- Gluon
- Goes
- gradually
- gravity
- grew
- Grows
- had
- Have
- he
- heavy
- High
- How
- However
- HTML
- HTTPS
- if
- in
- incorrect
- increased
- increasingly
- inevitably
- information
- inside
- interaction
- into
- involve
- involving
- isolated
- isolation
- issue
- IT
- jpg
- large
- lead
- likely
- LIMIT
- Low
- Main
- make
- many
- massive
- matt
- Matter
- max-width
- mean
- measuring
- mechanism
- member
- model
- models
- more
- most
- moving
- National
- neutrons
- never
- New
- North
- north carolina
- Notes
- number
- numerous
- of
- off
- on
- ONE
- ones
- only
- or
- original
- originally
- Other
- otherwise
- out
- overlap
- pair
- pairs
- perhaps
- Physics
- Physics World
- Plasma
- plato
- Plato Data Intelligence
- PlatoData
- plays
- Point
- Predictions
- process
- produce
- Produced
- Production
- Products
- pronounced
- proportion
- proposed
- protons
- Quarks
- quite
- rapidly
- rather
- ratio
- ratios
- really
- reason
- Recovers
- relative
- require
- requires
- research
- researchers
- Results
- review
- Role
- same
- says
- scenario
- Second
- seen
- several
- should
- show
- shown
- simultaneously
- small
- So
- something
- sometimes
- standard
- start
- Story
- strength
- strong
- studied
- surrounded
- Systems
- takes
- team
- tells
- texas
- than
- that
- The
- their
- Them
- theoretical
- There.
- These
- they
- think
- this
- thousands
- three
- Through
- thumbnail
- time
- to
- together
- true
- turns
- two
- underlying
- Universal
- university
- unlike
- upgraded
- us
- use
- used
- Vacuum
- very
- was
- Way..
- weak
- when
- which
- WHO
- whole
- with
- Work
- working
- works
- world
- years
- You
- zephyrnet