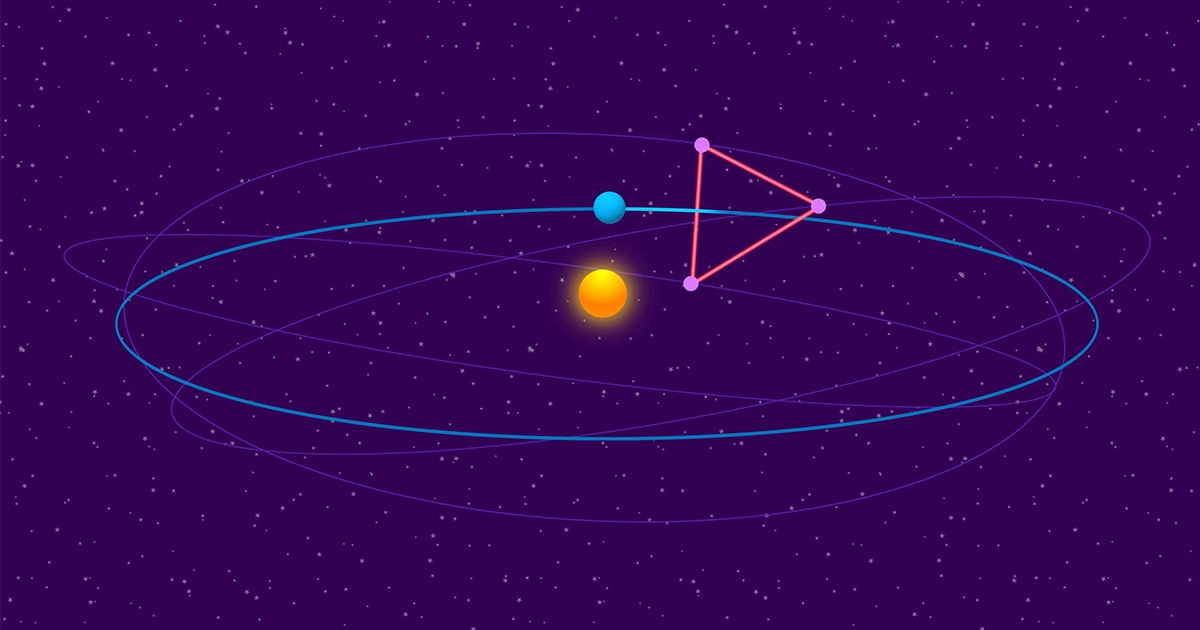
Introduction
At a conference in Japan a few years ago, David Dunsky attended a talk about gravitational waves, ripples in the fabric of space-time created when massive objects like stars and black holes accelerate.
Dunsky was a graduate student in particle physics at the time, and his interests seemingly lay elsewhere. Particle physicists seek the more fundamental truth underpinning the physical rules we’re familiar with. They’ve long used high-energy particle colliders to test their ideas. By smashing particles together at unfathomable energies, these scientists can discover the building blocks of the building blocks — the high-energy phenomena that happen at short distance scales. Those phenomena also tell us about the earliest moments of the universe when it was tiny, dense and incredibly hot.
But Dunsky learned at the talk that future gravitational wave observatories like the proposed Laser Interferometer Space Antenna (LISA) could be used to probe high-energy physics. LISA would be capable of detecting hypothetical objects called cosmic strings, vast strands of concentrated energy that might have arisen during the universe’s birth. “I got hooked on trying to understand gravitational wave signals from the early universe,” said Dunsky, who is now a cosmologist and particle physicist at New York University, “and how they could tell us about very, very high-energy physics potentially far beyond what we can currently detect with a collider.”
His turn toward gravitational waves as a way forward for particle physics exemplifies a widening interest in the future LISA experiment and, perhaps, a broader shift. Twelve years have passed since the last major discovery at a particle collider. The discovery of the Higgs boson at the Large Hadron Collider (LHC) in 2012 completed the Standard Model of particle physics, the reigning theory of the known elementary particles and forces. And while theorists have since thought up a zoo of possible theories extending the Standard Model, it’s not clear that we can build colliders capable of testing these ideas.
“People are talking about building colliders in the next 50 years that are 10 times more powerful than the LHC in terms of energy,” said Raman Sundrum, a theoretical particle physicist at the University of Maryland. However, testing grand unified theories, which trace the three forces of the Standard Model to a single underlying force operating at shorter distances, “would seem to take a collider which has 10 billion times the energy of the LHC,” he said.
What we can’t produce in a collider, we might be able to observe in nature. Specifically, answers might lie in the gravitational echoes of processes that unfolded in the first moments of creation, when the universe was so energetic that physics beyond the Standard Model would have reigned.
That’s the hope of particle physicists like Dunsky and Sundrum, who are now looking to LISA to test their theories. The mission concept was first developed in the early 1980s and formally proposed to the European Space Agency (ESA) the following decade. The project was pursued in collaboration with NASA for a time, but the Americans bowed out in 2011 due to budget concerns, forcing Europe to go it alone. This January, however, LISA finally got the go-ahead from ESA, which is now finding industry partners in order to begin construction. The announcement comes after the resounding success in 2015 and 2016 of a pilot mission, LISA Pathfinder, which tested the future observatory’s key technologies.
LISA is now scheduled to fly in the 2030s. For four years, its array of three satellites will tumble through space in an equilateral triangle some million miles across, bouncing lasers off the golden cubes kept in perfect free fall within each craft to feel for ripples in space-time.
“For the first time, we might actually get something directly from that very early epoch” of the universe, said Isabel Garcia Garcia, a particle physicist and cosmologist at the University of Washington. If LISA really can pick up primordial gravitational waves, she added, it will be our first glimpse of the cosmos’s first moments. “From the point of view of particle physics, that is obviously incredibly exciting.”
Lucky LISA
If indeed LISA manages to detect primordial gravitational waves sometime next decade, it will be due to a stroke of extraordinary cosmic luck.
No telescope will ever reveal the first moments of creation. Telescopes see into the universe’s past by detecting light that’s traveled from far away. But the first 380,000 years after the Big Bang are hidden behind a sort of cosmic curtain. Back then, the universe was filled with ionized plasma that scattered photons, making it opaque to light.
Unlike light, gravitational waves could ripple freely through the early universe. Existing ground-based observatories such as LIGO and Virgo probably aren’t sensitive to these primordial waves. But LISA might be able to hear what happened onstage before the cosmic curtain rose.
“It’s like hearing something in the fog,” Sundrum said.
Like ground-based gravitational wave observatories, LISA will detect ripples in space-time by using lasers to precisely measure the distance along its “arms” — in this case, the lines in empty space between the three spacecraft in its triangular constellation. When a gravitational wave passes by, it stretches and contracts space-time. This creates a slight difference in LISA’s arm lengths, which the instrument can detect by tracking the misalignment of the peaks and troughs of its laser beams. Removed from Earth’s noisy environment, LISA will be far more sensitive than existing interferometers like LIGO, which has been used to detect black hole and neutron star collisions. It will also be far larger; each of its arms will be nearly 400 times longer than the radius of the Earth.
Introduction
Even so, the changes in distance LISA will feel for are extremely small — about 50 times smaller than an atom. “It’s quite a crazy concept, if you think about it,” said Nora Lützgendorf, an astrophysicist at ESA and a LISA project scientist.
LISA’s size and sensitivity will allow it to observe gravitational waves that are much longer than those observable by ground-based interferometers. LIGO can sense gravitational waves with wavelengths between about 30 and 30,000 kilometers, but LISA can pick up waves ranging in length from a few hundred thousand kilometers to a few billion. This will let LISA listen in on astrophysical events that ground-based observatories can’t “hear,” such as the mergers of supermassive black holes (as opposed to star-sized black holes). And LISA’s wavelength band also happens to be exactly the size that physicists expect from gravitational waves generated in the first moments after the Big Bang.
High-energy physics in the early universe created gravitational ripples, and as the universe expanded and space stretched, these waves got blown up to enormous dimensions. LISA just happens to be perfectly poised to catch waves created in the first 10−17 to 10−10 seconds after the Big Bang — practically at the beginning of time. The short end of that range, 10−17 seconds, is a period so brief that it would fit about as many times into a second as seconds fit into the age of the universe.
“There is this serendipity,” said Chiara Caprini, a theoretical cosmologist at the University of Geneva and CERN. There’s a match between “the frequency band of detection of LISA and this particular epoch in the evolution of the universe which marks the frontier of our knowledge of particle physics.”
Beyond the Standard Model
Up to that frontier, the Standard Model does an excellent job of explaining how its flock of 17 elementary particles interact with three forces: the electromagnetic force, the strong nuclear force and the weak nuclear force. But despite its enormous successes, no one thinks that these particles and forces are the be-all and end-all of existence.
Introduction
The theory has its flaws. For instance, the mass of the Higgs boson — the component of the Standard Model that determines the masses of other particles — is frustratingly “unnatural.” It appears arbitrary, and puzzlingly small compared to the far greater energy scales of the universe. Moreover, the Standard Model offers no explanation for dark matter, nor for the mysterious dark energy that drives the accelerating expansion of space. Another problem is that antimatter and matter behave exactly the same under the three forces of the Standard Model — which obviously isn’t the full story, since matter dominates the universe. And then there’s gravity. The Standard Model completely ignores the fourth fundamental force, which must be described using its own bespoke theory, general relativity.
“So a lot of theorists like me have been trying to squeeze the Standard Model a little bit and try to make extensions of it,” said Pierre Auclair, a theoretical cosmologist at the Catholic University of Louvain in Belgium. But without experimental evidence with which to test them, these extended theories remain, well, theoretical.
Auclair is a theorist. “But still, I’m trying to be linked with experiments as much as I can,” he said. That’s one reason he was drawn to LISA. “These extensions usually lead to different extreme events in the early universe,” he said.
Garcia Garcia likewise said that LISA’s promise of observational evidence for high-energy physics led her to rethink her career — gravitational waves could “probe the early universe in a way no other experiment can,” she said. A few years ago, she started studying gravitational waves and how physics beyond the Standard Model would leave fingerprints detectable by LISA.
Last year, Garcia Garcia and her colleagues published work on the gravitational wave signature of bubble walls — energetic barriers between pockets of space that got trapped in different states as the universe cooled. This cooling happened as the universe expanded. Just as water boils and turns into steam, the universe went through phase transitions. In the Standard Model, the phase transition during which a single, “electroweak” force split into separate electromagnetic and weak forces was relatively smooth. But many extensions of the theory predict violent events that left the cosmic soup frothy and disturbed, said Dunsky, who also studies topological defects like bubble walls.
Quantum fields that permeate our universe have minimum-energy states, or ground states. And as the universe cooled down, new, lower-energy ground states developed, but a given field didn’t always immediately land in its new ground state. Some got trapped in local energy minima — false ground states that only appear stable. Sometimes, though, one little piece of the universe would quantum-tunnel into the true state, nucleating a rapidly expanding bubble of true vacuum with a lower energy than the universe outside.
“These bubbles are very energetic; they’re moving very close to the speed of light due to this pressure difference between their interior and exterior,” Dunsky said. “So when they collide, you get this violent collision between these two very relativistic objects, somewhat similar to how black holes emit strong gravitational waves right before colliding.”
Strings and Walls
More speculatively, phase transitions in the early universe could also have created structures called cosmic strings and domain walls — enormous strands and sheets, respectively, of dense energy.
These structures arise when a quantum field’s ground state changes in such a way that there is more than one new ground state, each equally valid. This can result in high-energy defects along the borders between pockets of the universe that happened to fall into different, but equally favorable, ground states.
The process is a bit like the way certain rocks develop natural magnetism as they cool, said Dunsky, who has studied the observable fingerprints of the process. At high temperatures, atoms are randomly oriented. But at cool temperatures, it becomes energetically favorable for them to magnetically align — the ground state changes. Without some external magnetic field to orient the atoms, they’re free to line themselves up any which way. All “choices” are equally valid, and different domains of the mineral will, by chance, make different choices. The magnetic field generated by all the atoms bends dramatically at the borders between domains.
Similarly, the quantum fields in different regions of the universe “must change rapidly at the boundary” of these domains, he said, resulting in large energy densities at these boundaries that “signify the presence of a domain wall or cosmic string.”
These cosmic strings and domain walls, if they exist, would have stretched out to span practically the entire universe as space expanded. These objects produce gravitational waves as kinks propagate along them and as loops oscillate and form cusps. But the energy scales of these waves were mostly set as the objects formed in the first moments of the universe. And LISA could detect them, if they exist.
Echoes of Creation
The gravitational waves reaching us from the very early universe will not arrive in neatly packaged chirps, like the signals of black hole collisions. Because they happened so early in time, such signals have since been stretched out across all of space. They’ll echo from every direction, from every point in space, all at once — a background gravitational hum.
“You turn on your detector, and it’s always there,” Garcia Garcia said.
Patterns in this background would probably “just look like noise to the average person,” Sundrum said. “But secretly, there’s a hidden code.”
One important clue will be the background signal’s spectrum — its strength at different frequencies. If we think of a gravitational wave signal as sound, its spectrum would be a plot of pitch versus volume. Truly random white noise would have a flat spectrum, Auclair said. But gravitational waves unleashed during phase transitions or cast from cosmic strings or domain walls would be loudest at specific frequencies. Auclair has worked on calculating the spectral signatures of cosmic strings, which throw out gravitational waves at characteristic wavelengths when their kinks and loops evolve. And Caprini studies how violent phase transitions would leave their own mark on the gravitational wave background.
Another approach, which Sundrum and his colleagues outlined in 2018 and recently elaborated, would be to try to map the overall intensity of the background across the sky. This would make it possible to look for anisotropies, or patches that are just a tiny bit louder or quieter than average.
“The problem,” Caprini said, “is that this kind of signal has practically the same characteristics of the instrument noise. So the entire question is how to be able to distinguish it once we detect something.”
LISA is more like a microphone than a telescope. Instead of peering in a particular direction, it will listen to the whole sky at once. It will hear primordial gravitational waves if they are present. But it will also hear the chirps and howls of merging black holes, neutron stars and the many pairs of white dwarf stars within our galaxy. In order for LISA to detect a background of primordial gravitational waves, all other signals will need to be carefully identified and removed. Filtering out the true signal from the early universe will be like picking out the sound of a spring breeze at a construction site.
But Sundrum chooses to be hopeful. “We’re not crazy to be doing the research,” he said. “It’ll be hard for experimentalists. It’ll be hard for the public to pay for the various things that need to get done. And it’ll be hard for theorists to calculate their way past all of the uncertainties and errors and backgrounds and so on.”
But still, Sundrum added, “it appears to be possible. With a little luck.”
- SEO Powered Content & PR Distribution. Get Amplified Today.
- PlatoData.Network Vertical Generative Ai. Empower Yourself. Access Here.
- PlatoAiStream. Web3 Intelligence. Knowledge Amplified. Access Here.
- PlatoESG. Carbon, CleanTech, Energy, Environment, Solar, Waste Management. Access Here.
- PlatoHealth. Biotech and Clinical Trials Intelligence. Access Here.
- Source: https://www.quantamagazine.org/hopes-of-big-bang-discoveries-ride-on-a-future-spacecraft-20240417/
- :has
- :is
- :not
- ][p
- $UP
- 000
- 10
- 121
- 17
- 2011
- 2012
- 2015
- 2016
- 2023
- 30
- 400
- 50
- 50 Years
- a
- Able
- About
- about IT
- accelerate
- accelerating
- across
- actually
- added
- After
- age
- agency
- ago
- align
- All
- allow
- alone
- along
- also
- always
- Americans
- an
- and
- Announcement
- Another
- answers
- Antimatter
- any
- appear
- appears
- approach
- arbitrary
- ARE
- arise
- ARM
- arms
- Array
- AS
- At
- atom
- average
- away
- back
- background
- backgrounds
- BAND
- barriers
- BE
- be-all
- because
- becomes
- been
- before
- begin
- Beginning
- behind
- Belgium
- bespoke
- between
- Beyond
- Big
- Big Bang
- Billion
- birth
- Bit
- Black
- Black Hole
- black holes
- Blocks
- borders
- boson
- boundaries
- breeze
- broader
- bubble
- budget
- build
- Building
- but
- by
- calculate
- calculating
- called
- CAN
- capable
- Career
- carefully
- case
- Catch
- certain
- Chance
- change
- Changes
- characteristic
- characteristics
- choices
- chooses
- clear
- Close
- code
- collaboration
- colleagues
- Collide
- collision
- comes
- compared
- Completed
- completely
- component
- Concentrated
- concept
- Concerns
- Conference
- construction
- contracts
- Cool
- Cosmos
- could
- craft
- crazy
- created
- creates
- creation
- Currently
- curtain
- Dark
- Dark matter
- decade
- described
- Despite
- detect
- Detection
- determines
- develop
- developed
- difference
- different
- dimensions
- direction
- directly
- discover
- discovery
- distance
- distinguish
- does
- doing
- domain
- domains
- dominates
- done
- down
- dramatically
- drawn
- drives
- due
- during
- each
- earliest
- Early
- Early Universe
- earth
- echo
- echoes
- elsewhere
- end
- energetic
- energy
- enormous
- Entire
- Environment
- epoch
- equally
- Errors
- ESA
- Europe
- European
- European Space Agency
- events
- EVER
- Every
- evidence
- evolution
- evolve
- exactly
- excellent
- exciting
- exemplifies
- exist
- existence
- existing
- expanded
- expanding
- expansion
- expect
- experiment
- experimental
- experiments
- explaining
- explanation
- extended
- extending
- extensions
- external
- extraordinary
- extreme
- extremely
- fabric
- Fall
- false
- familiar
- far
- favorable
- feel
- few
- field
- Fields
- filled
- filtering
- Finally
- finding
- First
- first time
- fit
- flat
- flaws
- Flock
- Fog
- following
- For
- Force
- Forces
- forcing
- form
- Formally
- formed
- Forward
- four
- Fourth
- Free
- freely
- Frequency
- from
- Frontier
- full
- fundamental
- future
- Galaxy
- General
- generated
- Geneva
- get
- given
- Glimpse
- Go
- Golden
- got
- graduate
- grand
- gravitational
- Gravitational waves
- gravity
- greater
- Ground
- happen
- happened
- happens
- Hard
- Have
- he
- hear
- hearing
- her
- Hidden
- High
- his
- Hole
- Holes
- hope
- hopeful
- hopes
- HOT
- How
- How To
- However
- HTML
- HTTPS
- hundred
- i
- ideas
- identified
- if
- immediately
- important
- in
- incredibly
- indeed
- industry
- industry partners
- instance
- instead
- instrument
- interact
- interest
- interests
- interior
- into
- IT
- ITS
- January
- Japan
- Job
- just
- kept
- Key
- kilometers
- Kind
- knowledge
- known
- Land
- large
- larger
- laser
- lasers
- Last
- lay
- lead
- learned
- Leave
- Led
- left
- Length
- let
- lie
- light
- like
- Line
- lines
- linked
- listen
- little
- local
- Long
- longer
- Look
- look like
- looking
- Lot
- louder
- lower
- luck
- magazine
- Magnetic field
- Magnetism
- major
- make
- Making
- manages
- many
- map
- mark
- Maryland
- masses
- massive
- Match
- Matter
- me
- measure
- mergers
- merging
- microphone
- might
- million
- mineral
- Mission
- model
- Moments
- more
- Moreover
- mostly
- moving
- much
- must
- Nasa
- Natural
- Nature
- nearly
- Need
- Neutron star
- Neutron stars
- New
- New York
- next
- no
- Noise
- now
- nuclear
- NYU
- objects
- observational
- observe
- of
- off
- Offers
- on
- once
- ONE
- only
- onstage
- opaque
- operating
- opposed
- or
- order
- Other
- our
- out
- outside
- overall
- own
- packaged
- pairs
- particular
- partners
- passed
- passes
- past
- Patches
- Pay
- perfect
- perfectly
- perhaps
- period
- person
- phase
- Photons
- physical
- physicist
- Physics
- pick
- picking
- piece
- Pierre
- pilot
- Pitch
- Plasma
- plato
- Plato Data Intelligence
- PlatoData
- plot
- pockets
- Point
- Point of View
- poised
- possible
- potentially
- powerful
- practically
- precisely
- predict
- presence
- present
- pressure
- probably
- probe
- Problem
- process
- processes
- produce
- project
- promise
- proposed
- public
- Quantamagazine
- Quantum
- question
- quite
- random
- range
- ranging
- rapidly
- reaching
- really
- reason
- regions
- relatively
- relativity
- remain
- Removed
- research
- resounding
- respectively
- result
- resulting
- reveal
- Ride
- right
- Ripple
- ripples
- ROSE
- rules
- Said
- same
- satellites
- scales
- scattered
- scheduled
- Scientist
- scientists
- Second
- seconds
- see
- Seek
- seem
- seemingly
- sense
- sensitive
- Sensitivity
- separate
- set
- she
- sheets
- shift
- Short
- shorter
- Signal
- signals
- signature
- Signatures
- similar
- since
- single
- site
- Size
- Sky
- small
- smaller
- smooth
- So
- some
- something
- sometimes
- somewhat
- Sound
- soup
- Space
- span
- specific
- specifically
- Spectral
- Spectrum
- speed
- split
- spring
- Squeeze
- stable
- standard
- Star
- Stars
- started
- State
- States
- Steam
- Still
- Story
- Strands
- strength
- String
- strong
- structures
- Student
- studies
- Studying
- success
- successes
- such
- Take
- Talk
- talking
- Technologies
- telescope
- telescopes
- tell
- terms
- test
- tested
- Testing
- than
- that
- The
- The Future
- their
- Them
- themselves
- then
- theoretical
- theory
- There.
- These
- they
- things
- think
- Thinks
- this
- those
- though?
- thought
- thousand
- three
- Through
- throw
- time
- times
- to
- together
- toward
- Trace
- Tracking
- transition
- transitions
- trapped
- traveled
- true
- truly
- truth
- try
- trying
- tumble
- TURN
- turns
- twelve
- two
- uncertainties
- under
- underlying
- underpinning
- understand
- unified
- Universe
- university
- unleashed
- us
- used
- using
- usually
- Vacuum
- valid
- various
- Vast
- Versus
- very
- View
- volume
- Wall
- was
- washington
- Water
- Wave
- waves
- Way..
- we
- weak
- web
- webp
- WELL
- went
- were
- What
- when
- which
- while
- white
- White Dwarf
- WHO
- whole
- widening
- will
- with
- within
- without
- worked
- would
- year
- years
- york
- You
- Your
- zephyrnet
- ZOO